Sea level rise will double coastal flood risk worldwide, according to research, published in the Scientific Reports journal.
It is the first to analyse coastal flood factors, particularly waves, on a global scale and found that the most at-risk areas were in the low latitudes, where tidal ranges are smaller meaning sea level rise is proportionally more significant.
Abstract
Introduction
Coastal regions experience elevated water levels on an episodic basis due to wave setup and runup8, tides9, storm surge driven by wind stress and atmospheric pressure, contributions from seasonal and climatic cycles, e.g., El Niño/Southern Oscillation10, 11 and Pacific Decadal Oscillation12, and oceanic eddies13 (Fig. 1).
Coastal flooding often occurs during extreme water-level events that result from simultaneous, combined contributions, such as large waves, storm surge, high tides, and mean sea-level anomalies11, 14.
SLR leads to (1) passive high-tide inundation of low-lying coastal areas15, (2) increased frequency, severity, and duration of coastal flooding16, (3) increased beach erosion17, (4) groundwater inundation18, 19, (5) changes to wave dynamics20, and (6) displacement of communities21. Predicting regions vulnerable to passive inundation is relatively simple with the aid of high-resolution digital elevation models22. However, predicting the effect of SLR on episodic flooding events is difficult due to the unpredictable nature of coastal storms, nonlinear interactions of physical processes (e.g., tidal currents and waves), and variations in coastal geomorphology (e.g., sediments, bathymetry, topography, and bed friction). Local-scale assessments of coastal hazard vulnerability typically rely on detailed, computationally-onerous numerical modeling efforts23 in order to simulate wave-related nearshore water levels, interactions with local topography, and the resulting flooding. Global-scale coastal hazard vulnerability assessments, on the other hand, rely on extreme value theory applied to water-level observations.
Extreme-value theory
Extreme-value theory24, 25 is a statistical method for quantifying the probability or return period of large events. The generalized extreme value (GEV) distribution, sometimes called the Fisher-Tippet distribution, is a powerful and general statistical model for extremes26 (Coles 2001). The GEV distribution models the probabilities of the maxima of a random variable24, 27, 28 using three parameters μ, σ, and k, the location (mean), scale (width), and shape (family type), respectively26.
Oceanographic and coastal engineering studies often rely on GEV theory to describe the frequency of extreme waves29, water-level events30, flooding impacts31, and to understand the effects of SLR32. As sea level increases, the probability increases that a fixed elevation will experience flooding (Fig. 2). Equivalently, the return period or recurrence interval of flooding at a fixed elevation decreases33, 34. In the example shown in Fig. 2B, 1 m of SLR causes the 5 m flood level (the former 100-year flood) to recur every 25 years.
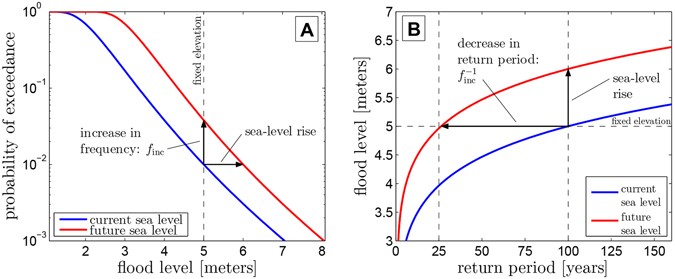
SLR can affect flood magnitude and frequency directly (Fig. 2) or indirectly via hydrodynamic feedbacks: SLR alters water depths, changing the generation, propagation, and interaction of waves, tides, and storm surges. Thus, SLR and long-term changes in wave climate, e.g., changes in magnitude, frequency, and tracks of storms35,36,37 and storm surge, can alter the parameters of extreme water-level distributions and the evolution of coastal hazards over time. In the proposed work, we assume parameter stationarity based on projections of minor changes (5–10%35,36,37) in mean annual wave conditions and storm surge over large regions of the ocean. In specific locations, such as the Pacific Northwest, trends in extreme wave climate may be significant38 and lead to a greater flooding hazard than SLR over at least the next several decades39, calling for nonstationary methods40 in future research.
Investigations of increased flooding frequency due to SLR are often site-specific and rely only on water-level data from tide stations. For example, Hunter (2012) [ref. 41] and the Intergovernmental Panel on Climate Change (IPCC) 2013 report3 estimate the factor of increase in the frequency of flooding events due to 0.5 m of SLR at locations of 198 tide stations around the globe [Hunter41 Fig. 4 and IPCC3 Fig. 13.25]. Hunter41 and IPCC3 found that regions with low variability of extreme water levels will experience large increases in flooding frequency. This finding, introduced qualitatively by Hoozemans et al. [ref. 33], is critical to predict the global regions most vulnerable to SLR. However, global-scale coastal hazard assessments using this methodology encounter three challenges: (1) Water-level observation stations are sparsely located around the globe, especially in the Indian Ocean and South Atlantic; (2) wave-driven water-level contributions, i.e., setup and swash, are not included; and (3) the global variability of the GEV shape parameter has not been considered, although it can be as influential as the scale parameter in determining vulnerability. Here we meet the three challenges by using extreme-value theory to combine sea level, wave, tide, and storm-surge models to predict increases in extreme water-level frequency on a global scale.
Application
Flooding results from the complex interaction of extreme water levels, topography, and the built environment. Here we use the frequency of extreme water levels as a proxy for regional-scale increases in flooding frequency, while recognizing that the relationship between water level and flooding is location dependent because of coastal topography, coastal defense structures, and drainage systems.
We apply sea-level projections and global wave, tide, and storm surge models to predict the future return periods (associated with the former 50-yr extreme water level) due to SLR. As in Hunter41 and IPCC3, we begin by investigating increases in flooding frequency due to a globally-uniform amount of SLR, acknowledging that spatial variability in the regional rate of SLR (e.g., driven by ocean circulation patterns, glacial fingerprinting) and the local relative rate of SLR (e.g., due to tectonic activity, glacial isostasy, land subsidence) will affect flooding predictions for specific locations42. Later we take the inverse approach, estimating the amount of SLR that doubles the frequency of extreme water-level events.
Using maximum likelihood estimates, we fit GEV probability distributions to the top three annual maximum water-level events from 1993–2013 obtained via synthesis of the Global Ocean Wave (GOW) reanalysis43, Mog2D storm-surge model44, and TPXO tide model45 as discussed in Methods. Figure 3 shows the global variability of the mean (μ), scale (σ), and shape (k) parameters for extreme total water level in panels A, B, and C, respectively. The GEV parameters provide necessary inputs to the factors of increase, f inc , and the future return period of the former 50-yr water level based on Eq. (3) (see Methods). Figure 4 shows the factor of increase for the SLR projections μ SL = +0.1, +0.25, +0.5 m on a global scale. Finally, the GEV parameters allow for global estimation of the amount of SLR that doubles the exceedance probability of the 50-yr water-level elevation [see Fig. 5 and Methods Eq. (4)]. Analyzing the amount of SLR leading to a doubling in flooding (Fig. 5) is equivalent to the factor-of-increase results shown in Fig. 4, but it provides a more intuitive picture of the effects of small amounts of SLR. Table 1 summarizes the global, tropical, and extra-tropical mean values of the quantities presented in Figs 3 and 5. Although the plotted distributions apply only to coasts, they are calculated ocean-wide in order to reveal the continuous global pattern of vulnerability of both continental coastal settings and non-contiguous island nations throughout the world’s oceans.
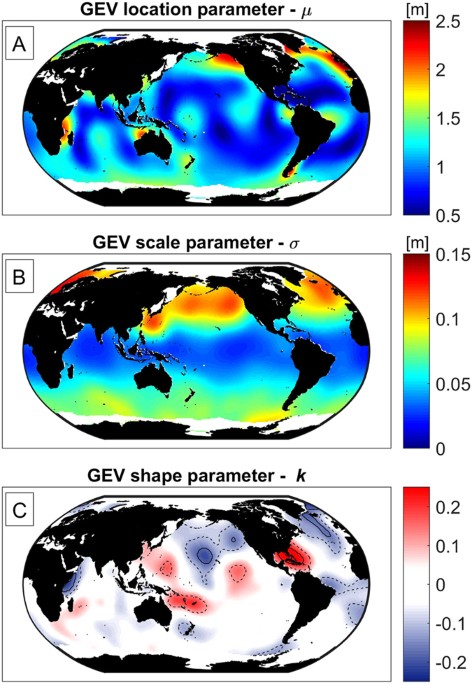
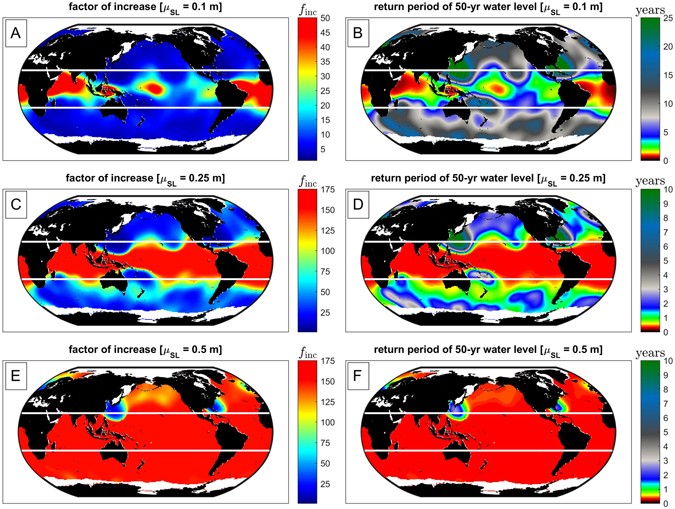
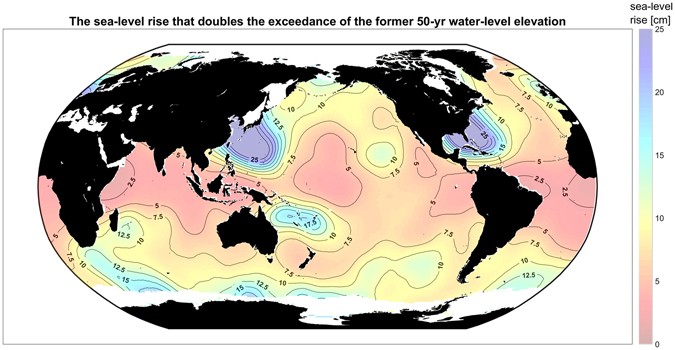
Source: https://www.nature.com/articles/s41598-017-01362-7